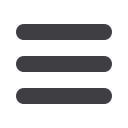
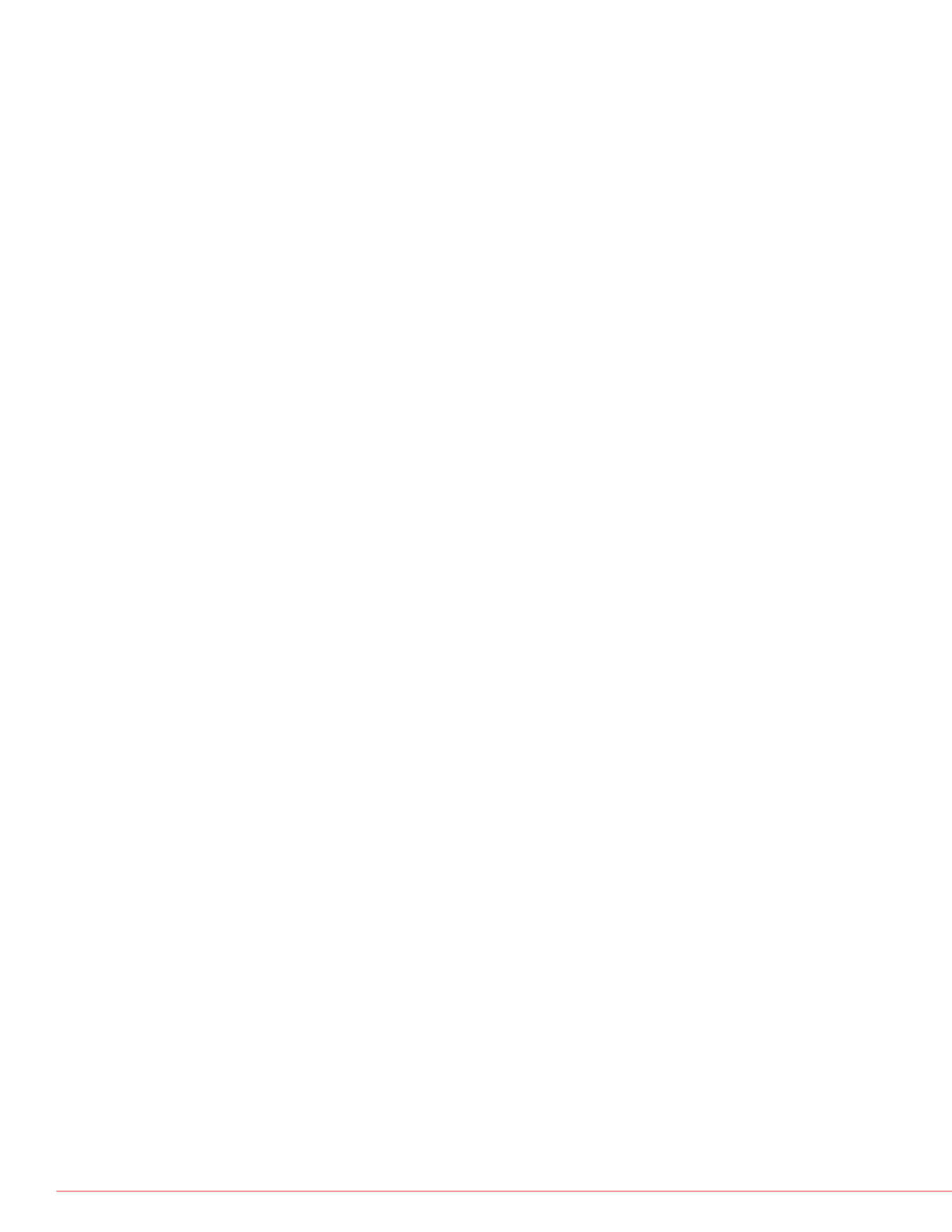
2
Improving Throughput for Highly Multiplexed Targeted Quanti cation Methods Using Novel API-Remote Instrument Control and State-Model
Data Acquisition Schemes
Overview
Automated remote multiplexed targeted protein quantification utilizing real-time
qual/quan processing for increased quantitative accuracy over large dynamic ranges.
Introduction
Targeted quantification has become a very popular technique to verify putative
biomarker candidates in large clinical cohorts of samples. These candidates are
usually generated following a biomarker discovery experiment or derived from a
biological hypothesis, for example, a pathway or biophysical interaction. These lists
are usually large, containing upwards of 100–1000 proteins spanning several orders of
magnitude dynamic concentration range. This presents analytical challenges for
conventional SRM assays both in terms of method development and throughput. We
propose using high-resolution, accurate-mass (HR/AM) mass spectrometry (MS) and
MS/MS schemes in conjunction with validated spectral libraries for automated method
building, data acquisition, verification, and quantification in real-time using novel
acquisition schemes.
Methods
K562 colon carcinoma cells were grown in heavy and light media, collected and mixed
at different ratios to cover a 20-fold dynamic range. All samples were digested and
analyzed on a quadrupole Thermo Scientific™ Orbitrap™ mass spectrometer
equipped with a nanospray ion source. Data was acquired in two steps to simulate
traditional workflows. Initial experiments employed unbiased data-dependent MS/MS
acquisition resulting in peptide/protein identification as well as building of a spectral
library. The spectral library contains relative retention time, precursor charge state
distribution, and product ion distributions, creating a unique verification/quantification
scheme. A highly multiplexed, targeted protein list was created from the spectral library
and used for automated data acquisition and processing real time to facilitate changes
to the acquisition scheme.
The scheme in Figure 1 describes the methodology in more detail. The first step is to
characterize the LCMS parameters using the PRTC kit. The next step is to build a list
of proteins that we are interested in. This will typically come from a pathway study or a
discovery experiment. The next is to build a spectral library for this list of proteins. This
can be built via predictive algorithm or empirical observations. This turns into a
spectral library lookup table. The look-up table includes the precursor
m/z
values for
the defined charge state as well as the expected retention time window, which are
used to initiate product ion spectral acquisition based on the presence of multiple
precursor isotopes during the expected elution window. Once the signal for multiple
precursor isotopes surpasses the user-defined intensity threshold, a higher-energy
collision dissociation (HCD) spectrum is acquired and immediately compared against
the spectral library generating a dot-product correlation coefficient to determine
spectral overlap and to check if the targeted peptide has been detected previously. If
the calculated correlation coefficient surpasses the user-defined acceptance value,
HCD product ion spectra will continue to be acquired across the elution profile. This is
shown in Figure 2.
FIGURE 2. Pictorial represen
targeted peptide quantificati
elution identification, and re
precursor and production s
selectivity of data acquisitio
FIGURE 1. Strategy for large
data acquisition scheme
*
*
Measured Ion Intensity
Start time for “watch
Triggerin
Threshol
1.
Theoretical
Isotope
Experimental
HR/AM MS
Spectrum
LC-MS characterization using the
to determine:
Scheduled retention time wind
Average chromatographic pea
Determine targeted protein list:
Discovery experiments
Pathway determination
Functional groups
State-model data acquisition:
Real-time data interrogation
Target peptide prioritization
On-the-fly data processing
Scheme